Where Does The Mass Of A Proton Come From?
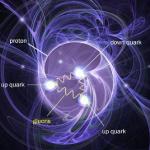
If you were to divide the particles that made up your body into smaller and smaller bits, you’d find that at every step along the way — at least in terms of mass — the whole was equal to the sum of its parts. If you broke your body down into its individual bones, fat and organs, they would add up to an entire human being. If you broke them down further, into cells, again, the cells would still add up to the same mass as you. Cells can be divided into organelles, organelles broken up into individual molecules, molecules into atoms, and atoms into protons, neutrons and electrons. At that level, there’s a tiny but noticeable difference: the individual protons, neutrons and electrons are off by right around 1% from a human, thanks to nuclear binding energy.
The nucleus of a carbon atom has a mass that’s approximately 0.8% lower than the individual protons and neutrons that compose it, thanks to nuclear binding energy. Image credit: Delia Walsh of http://slideplayer.com/slide/6002405/.
A carbon atom, made up of six protons and six neutrons, is approximately 0.8% lighter than the individual component particles that make it up. The way carbon is formed is through the nuclear fusion of hydrogen into helium and then helium into carbon; the energy released is what powers most types of stars in both their normal and red giant phases, and the “lost mass” is where that energy comes from, thanks to Einstein’s E = mc2. This is how most types of binding energy work: the reason it’s harder to pull apart multiple things that are bound together is because they released energy when they were joined, and you have to put energy in to free them again.
Which is why it’s such a puzzling fact that when you take a look at the particles that make up the proton — the three different quarks at the heart of them — their combined masses are only 0.2% of the mass of the proton as a whole.
The particles of the standard model, with masses (in MeV) in the upper right. A proton, made up of two up quarks and one down quark, has a mass of ~938 MeV/c^2. Image credit: Wikimedia Commons user MissMJ, PBS NOVA, Fermilab, Office of Science, United States Department of Energy, Particle Data Group, under a c.c.a.-3.0 unported license.
The way quarks bind into protons is fundamentally different from all the other forces and interactions we know of. Instead of the force getting stronger when objects get closer — like the gravitational, electric or magnetic forces — the attractive force goes down to zero when quarks get arbitrarily close. And instead of the force getting weaker when objects get farther away, the force pulling quarks back together gets stronger the farther away they get.
This property of the strong nuclear force is known as asymptotic freedom, and the particles that mediate this force are known as gluons. Somehow, the energy binding the proton together, the other 99.8% of the proton’s mass, comes from these gluons.
Rather than three main, green (valence) quarks connected by (spring-like) gluons, the proton’s structure is much more complicated, with additional (sea) quarks and gluons populating the proton’s interior. Image credit: the German Electron Synchrotron (DES), and the HERA and ZEUS collaborations.
Because of how the strong nuclear force works, there are large uncertainties as to where these gluons are actually located at any point in time. We presently have a solid model of the average gluon density inside a proton, but if we want to know where the gluons are actually more likely to be located, that requires more experimental data, as well as better models to compare the data against. Recent advances by theorists Björn Schenke and Heikki Mäntysaari may be able to provide those much needed models. As Mäntysaari detailed:
It is very accurately known how large the average gluon density is inside a proton. What is not known is exactly where the gluons are located inside the proton. We model the gluons as located around the three quarks. Then we control the amount of fluctuations represented in the model by setting how large the gluon clouds are, and how far apart they are from each other.
The internal structure of a proton, with quarks, gluons, and quark spin shown. Image credit: Brookhaven National Laboratory.
When you collide two particles like protons, a proton and a heavy ion, or two heavy ions together, you can’t simply model them as proton-proton collisions. Instead, you see a distribution of three types of collisions: quark-quark collisions, quark-gluon collisions or gluon-gluon collisions. It’s the components within these subatomic particles that actually collide, rather than the entire structures (the protons) themselves. While at lower energies, it’s almost always quarks that collide, the higher energies reached by RHIC, the Relativistic Heavy Ion Collider, at Brookhaven and by the LHC at CERN have a very high probability of gluon-gluon interactions, with the potential to reveal the location of the gluons inside a proton itself. As Mäntysaari continued:
This process doesn’t happen at all if the proton always looks the same. The more fluctuations we have, the more likely this process is to happen.
A better understanding of the internal structure of a proton, including how the “sea” quarks and gluons are distributed, has been achieved through both experimental improvements and new theoretical developments in tandem. Image credit: Brookhaven National Laboratory.
The combination of this new theoretical model and the ever-improving LHC data will better enable scientists to understand the internal, fundamental structure of protons, neutrons and nuclei in general, and hence to understand where the mass of the known objects in the Universe comes from. The biggest boon to this type of research, however, would be the development of an Electron-Ion Collider (EIC), a proposed collider by many collaborations across the world. Unlike RHIC or the LHC, which collide protons with ions — resulting in a very messy final signal — an EIC would be much more controlled, as there are no internal, uncontrollable motions inside an electron to confound the experimental results.
A schematic of the world’s first electron-ion collider (EIC). Adding an electron ring (red) to the Relativistic Heavy Ion Collider (RHIC) at Brookhaven would create the eRHIC. Image credit: Brookhaven National Laboratory-CAD eRHIC group.
If you want to study the internal structure of a proton or collection of nuclei, deep inelastic scattering is the only way to go. Considering that colliders began that journey less than a century ago, and that we’re now achieving energies approximately a factor of 10,000 greater than when we first started, probing and understanding exactly how matter gets its mass may finally be within our reach. The quark-gluon plasma within the nucleus, and the attendant fluctuations, may finally be ready to reveal its secrets to us. And when it does, one of the longest-standing mysteries of physics, of where the mass of the known matter comes from (still a mystery even after discovery of the Higgs), may finally yield to humanity.
Astrophysicist and author Ethan Siegel is the founder and primary writer of Starts With A Bang. Follow him on Twitter, Facebook, G+, Tumblr, and order his book: Beyond The Galaxy, today!