Simple Bacteria Offer Clues to the Origins of Photosynthesis
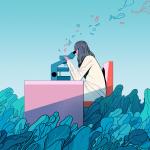
Researchers have caught their best glimpse yet into the origins of photosynthesis, one of nature’s most momentous innovations. By taking near-atomic, high-resolution X-ray images of proteins from primitive bacteria, investigators at Arizona State University and Pennsylvania State University have extrapolated what the earliest version of photosynthesis might have looked like nearly 3.5 billion years ago. If they are right, their findings could rewrite the evolutionary history of the process that life uses to convert sunlight into chemical energy.
Photosynthesis directly or indirectly powers and sustains almost every organism on Earth. It is responsible for the composition of our atmosphere and forms the foundation of the planet’s many interwoven ecosystems. Moreover, as Wolfgang Nitschke, a biologist at the French National Center for Scientific Research (CNRS) in Paris, noted, photosynthesis liberated cells to grow and evolve boundlessly by letting them derive energy from a new, inexhaustible, nonterrestrial source. “When photosynthesis entered the picture, life connected up to the cosmos,” he said.
Scientists want to figure out what made that possible. In its current form, the machinery that converts light energy to chemical energy in photosynthesis — a protein complex called a reaction center — is incredibly sophisticated. The evidence suggests, however, that its design, which stretches back almost to the root of the tree of life, was once very simple. Researchers have been trying for decades to fill that enormous gap in their understanding of how (and why) photosynthesis evolved.
To that end, they have turned their attention to existing organisms. By studying the molecular details of the reactions that green plants, algae and some bacteria use to photosynthesize, and by analyzing the evolutionary relationships among them, scientists are trying to piece together a cogent historical narrative for the process.
The muddy soils around geothermal hot springs in Iceland, like the Geysir spring pictured here, are the natural habitat for primitive photosynthetic heliobacteria. Scientists are now studying those organisms for insights into the early evolution of photosynthesis. Vincent Moschetti
The latest important clue comes from Heliobacterium modesticaldum, which has the distinction of being the simplest known photosynthetic bacterium. Its reaction center, researchers think, is the closest thing available to the original complex. Ever since the biologists Kevin Redding, Raimund Fromme and Christopher Gisriel of Arizona State University, in collaboration with their colleagues at Penn State, published the crystallographic structure of that protein complex in a July edition of Science, experts have been unpacking exactly what it means for the evolution of photosynthesis. “It’s really a window into the past,” Gisriel said.
“This is something we’ve been waiting for for 15 years,” Nitschke said.
In Search of a Common Ancestor
At first, most scientists did not believe that all the reaction centers found in photosynthetic organisms today could possibly have a single common ancestor. True, all reaction centers harvest energy from light and lock it into compounds in a form that’s chemically useful to cells. To do this, the proteins pass electrons along a transfer chain of molecules in a membrane, as though skipping along a series of stepping stones. Each step releases energy that’s ultimately used down the line to make energy-carrier molecules for the cell.
But in terms of function and structure, the photosystem reaction centers fall into two categories that differ in almost every way. Photosystem I serves mainly to produce the energy carrier NADPH, whereas photosystem II makes ATP and splits water molecules. Their reaction centers use different light-absorbing pigments and soak up different portions of the spectrum. Electrons flow through their reaction centers differently. And the protein sequences for the reaction centers don’t seem to bear any relation to each other.
Both types of photosystem come together in green plants, algae and cyanobacteria to perform a particularly complex form of photosynthesis — oxygenic photosynthesis — that produces energy (in the form of ATP and carbohydrates) as well as oxygen, a byproduct toxic to many cells. The remaining photosynthetic organisms, all of which are bacteria, use only one type of reaction center or the other.
So it seemed as though there were two evolutionary trees to follow — that was, until the crystal structures of these reaction centers began to emerge in the early 1990s. Researchers then saw undeniable evidence that the reaction centers for photosystems I and II had a common origin. Specific working components of the centers seemed to have undergone some substitutions during evolution, but the overall structural motif at their cores was conserved. “It turned out that big structural features were retained, but sequence similarities were lost in the mists of time,” said Bill Rutherford, the chairman in biochemistry of solar energy at Imperial College London.
“Nature has played small games to change some of the functions of the reaction center, to change the mechanisms by which it works,” Redding added. “But it hasn’t rewritten the playbook. It’s like having a cookie-cutter design for a house, building that same house over and over again, and then changing how the rooms are arranged, how the furniture is positioned. It’s the same house, but the functions inside are different.”
Researchers began to make more detailed comparisons between the reaction centers, searching for clues about their relationship and how they diverged. Heliobacteria have brought them a few steps closer to that goal.
Harkening Back to an Earlier Time
Since it was discovered in the soil around Iceland’s hot springs in the mid-1990s, H. modesticaldum has presented researchers with an interesting piece of the photosynthesis puzzle. The only photosynthetic bacterium in a family with hundreds of species and genera, heliobacteria’s photosynthetic equipment is very simple — something that became even more apparent when it was sequenced in 2008. “Its genetics are very streamlined,” said Tanai Cardona, a biochemist at Imperial College London.
Robert Blankenship, a photosynthesis researcher at Washington University, looks at a flask of cultured cyanobacteria. The organizational simplicity of heliobacteria, he said, “harkens back to an earlier evolutionary time.”
Heliobacteria have perfectly symmetrical reaction centers, use a form of bacteriochlorophyll that’s different from the chlorophyll found in most bacteria, and cannot perform all the functions that other photosynthetic organisms can. For instance, they cannot use carbon dioxide as a source of carbon, and they die when exposed to oxygen. In fact, their structure took nearly seven years to obtain, partly because of the technical difficulties in keeping the heliobacteria insulated from oxygen. “When we first started working on it,” Redding said, “we killed it more than once.”
Taken together, “heliobacteria have a simplicity in their organization that’s surprising compared to the very sophisticated systems you have in plants and other organisms,” said Robert Blankenship, a leading figure in photosynthesis research at Washington University in St. Louis. “It harkens back to an earlier evolutionary time.”
Its symmetry and other features “represent something quite stripped down,” Redding added, “something we think is closer to what that ancestral reaction center would have looked like three billion years ago.”
A Glimpse of the Past
After carefully taking images of the crystallized reaction centers, the team found that although the reaction center is officially classified as type I, it seemed to be more of a hybrid of the two systems. “It’s less like photosystem I than we thought,” Redding said. Some people might even call it a “type 1.5,” according to Gisriel.
One reason for that conclusion involves greasy molecules called quinones, which help transfer electrons in photosynthetic reaction centers. Every reaction center studied so far uses bound quinones as intermediates at some point in the electron transfer process. In photosystem I, the quinones on both sides are tightly bound; in photosystem II, they are tightly bound on one side, but loosely bound on the other. But that’s not the case in the heliobacterium reaction center: Redding, Fromme and Gisriel did not find permanently bound quinones among the electron transfer chain’s stepping stones at all. That most likely means its quinones, although still involved in receiving electrons, are mobile and able to diffuse through the membrane. The system might send electrons to them when another, more energetically efficient molecule isn’t available.
Raimund Fromme, Christopher Gisriel and Kevin Redding (from left to right) are researchers in the School of Molecular Sciences at Arizona State University. With colleagues at Pennsylvania State University, they recently determined the crystallographic structure of the energy-producing reaction center in the simplest known photosynthetic bacterium. Arizona State University
This finding has helped the research team deduce what early reaction centers may have been doing. “Their job was likely to reduce mobile quinones,” Redding said. “But they weren’t doing a very good job of it.” In the researchers’ scenario, tightly bound quinone sites are a more recent adaptation, and today’s type I and type II reaction centers represent alternative evolutionary strategies, embraced by different lineages of organisms, for improving on the ancestral system’s sloppy, less-than-ideal work.
“But then the question is, why has nature changed this kind of electron transfer chain?” Fromme asked. His work supports the hypothesis that it might have something to do with oxygen.
When an organism is exposed to too much light, electrons build up in the transfer chain. If oxygen is around, this buildup can lead to a harmfully reactive oxygen state. Adding a firmly bound quinone to the complex not only provides an additional slot to deal with potential traffic jams; the molecule, unlike others used in the transfer chain, also does not pose any risk of producing that deleterious form of oxygen. A similar explanation works for why reaction centers became asymmetric, Gisriel added: Doing so would have added more stepping stones as well, which would have similarly buffered against damage caused by the accumulation of too many electrons.
One of the researchers’ next steps is to put time stamps on when this asymmetry and these tightly bound quinones came into the picture, which would help them determine when oxygenic photosynthesis became possible.
All Roads Lead to Oxygen
Cardona, who was not involved in the recent study but has begun interpreting its results, thinks he may have found a hint in the heliobacterium reaction center. According to him, the complex seems to have structural elements that would have later lent themselves to the production of oxygen during photosynthesis, even if that wasn’t their initial purpose. He found that a particular binding site for calcium in the heliobacteria’s structure was identical to the position of the manganese cluster in photosystem II, which made it possible to oxidize water and produce oxygen.
Tanai Cardona, a biochemist at Imperial College London, suspects that cells may have been producing oxygen through photosynthesis for about a billion years longer than scientists usually assume. Imperial College London
“If the ancestral site at some later stage turned into the manganese cluster,” Cardona said, “that would suggest that water oxidation was involved in the earliest events in the divergence between type I and type II reaction centers.” That, in turn, would mean oxygenic photosynthesis was far more ancient than expected. Scientists have commonly supposed that oxygenic photosynthesis appeared shortly before the Great Oxygenation Event, when oxygen began to build up in Earth’s atmosphere and caused a mass extinction 2.3 to 2.5 billion years ago. If Cardona is right, it may have evolved nearly a billion years earlier, shortly after photosynthesis made its debut.
That timing would have been early enough to predate the cyanobacteria typically credited as the first organisms to perform oxygenic photosynthesis. According to Cardona, it may be the case that a lot of bacteria could do it, but that after mutations, divergences and other events, only cyanobacteria retained the ability. (Cardona published a paper this year citing other molecular evidence for this hypothesis. He has not yet formally presented arguments about the potential link involving calcium for peer review, but he has written about the idea in blog posts on his website and on a scientific networking site for researchers, and he recently began working on a paper about it.)
That hypothesis contradicts one of the widely held ideas about the origins of photosynthesis: that species incapable of photosynthesis suddenly obtained the capacity through genes passed laterally from other organisms. According to Cardona, in light of the new discoveries, horizontal gene transfer and gene loss may both have played a role in the diversification of reaction centers, although he suspects that the latter may have been responsible for the earliest events. The finding, he said, might suggest that “the balance skews toward the gene-loss hypothesis” — and toward the idea that photosynthesis was an ancestral characteristic that some groups of bacteria lost over time.
Not everyone is so sure. Blankenship, for one, is skeptical. “I don’t buy that,” he said. “I don’t see any data here that suggests that oxygenic photosynthesis occurred that much earlier.” To him, the work by Redding, Fromme and their collaborators has not answered these questions; it has only conjectured about what may have happened. To solve that puzzle, scientists will need the reaction center structures of other bacteria, so they can continue evaluating the structural differences and similarities to refine the twisting roots of their evolutionary trees.
“I think it’s entirely a possibility that what is saying is correct,” Gisriel said, “but I also think the field should sit with it for a while, do some more analysis and see if we understand more about how this structure works.”
Going the Synthetic Route
Some researchers aren’t waiting for the publication of the next structure. This one took seven years, after all. They’re pursuing synthetic experimentation instead.
Rutherford and his colleagues, for example, are using a “reverse evolution” technique: They hope to predict the sequences of missing-link reaction centers, using structural information like Redding’s to gain an understanding of their architecture. They then plan to synthesize those hypothetical ancestral sequences and test how they evolve.
Meanwhile, Redding and his team have just begun artificially converting the symmetric reaction center of heliobacteria into an asymmetrical one, following in the footsteps of two researchers in Japan, Hirozo Oh-Oka of Osaka University and Chihiro Azai of Ritsumeikan University, who have spent more than a decade doing this in another type of photosynthetic bacterium. The groups believe their work will clarify how these adaptations would have occurred in real life in the distant past.
Twenty years ago, Nitschke stopped working on the evolution of photosynthesis and turned his attention to other problems. “It seemed so hopeless,” he said. But the research done by Redding, his team and these other groups has rekindled those ambitions. “As they say, your first love always stays with you,” Nitschke said. “I’m really excited about this new structure and plan to go back to thinking about all this again.”