Dark Energy Might Be Neither Particle nor Field
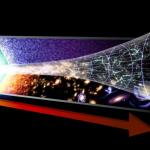
What is it, at a fundamental level, that makes up the universe? When we ask this question, we typically think about starting with things that we directly observe — things like stars, planets, humans, gas, dust, plasma, and other forms of the matter we know — and dividing them up until you reach something that is indivisible. Although we originally thought that atoms would be these “uncuttable” things, we soon discovered they could be further divided: into electrons and atomic nuclei, which themselves are composed of quarks and gluons.
As we mastered the laws of physics and began to manipulate these subatomic particles, we gained the ability to accelerate and collide them, enabling the creation of a wide slew of particles and antiparticles: everything described by the Standard Model of particle physics. And yet, if we add up the sum total of all of these forms of matter, including photons, neutrinos, and everything that does not compose atoms, we fall far short of what is needed to describe our universe. Two additional components are necessary: dark matter and dark energy. Moreover, although we fully expect there to be a particle responsible for dark matter, that is not the case at all for dark energy. Here’s why.
The particles and antiparticles of the Standard Model obey all sorts of conservation laws, with fundamental differences between fermionic particles and antiparticles and bosonic ones. Each set of particles possesses its own unique quantum numbers, but no particles here can explain dark matter or dark energy. (Credit: E. Siegel / Beyond the Galaxy)
Particles, at least as we know them, all have a few things in common. They have a set of “quantum numbers,” or properties that are inherent to them that determine their masses, spins, and various charges. All particles of the same type — electrons, down quarks, Z-bosons, etc. — have identical properties to one another, and you could replace any one of them with any other identical particle and everything would remain the same. The only things that differ between them are either random, like their decay lifetime (if they are unstable), or situational: things like their momentum, orbital angular momentum, or energy levels within a bound system.
But there is another way to break these various particles up: into massive and massless categories. Massive particles slow down as the universe expands and cools during the hot Big Bang and eventually gravitationally clump together, as every mass universally attracts every other mass. Massless particles, however, simply travel through curved space at the only permissible speed, the speed of light, until they interact with another particle in their path. Massive (matter) and massless (radiation) particles evolve in fundamentally different ways with respect to the expanding universe.
While matter and radiation become less dense as the universe expands owing to its increasing volume, dark energy is a form of energy inherent to space itself. As new space gets created in the expanding universe, the dark energy density remains constant. (Credit: E. Siegel / Beyond the Galaxy)
Astrophysically, when we survey the universe in all the different ways we know of, it reveals a variety of aspects of the cosmic story. By observing how abundant the lightest elements and their isotopes are, we can determine how much normal matter, total, makes up our universe. By measuring how galaxies clump and cluster together, as well as their large-scale distribution and individual, internal properties, we can determine how much total “stuff” there is that behaves as though it is made of massive particles. And when we look at the details embedded in the leftover glow from the Big Bang — the cosmic microwave background — it tells us that the universe is spatially flat, telling us how much total “stuff” is present in the universe, overall.
From this information, we learn that all of the normal, Standard Model material in our universe comes out to just 5 percent of the total. Another ~27 percent is dark matter, which cannot behave like any of the known particles. It clumps and gravitates like normal matter but appears to have zero cross-section — i.e., doesn’t collide — with normal matter, light, or other dark matter particles. Although we can only detect dark matter’s presence through its gravitational influence, it is immediately apparent that dark matter is distributed far more diffusely than normal matter; it isn’t as clumpy, particularly on small cosmic scales. Unfortunately, all attempts at direct detection experiments have failed to yield a robust, positive signal. Its true nature remains mysterious.
Light may be emitted at a particular wavelength, but the expansion of the universe will stretch it as it travels. Light emitted in the ultraviolet will be shifted all the way into the infrared when considering a galaxy whose light arrives from 13.4 billion years ago. The more the expansion of the universe accelerates, the greater the light from distant objects will be redshifted and the fainter it will appear. (Credit: Larry McNish / RASC Calgary)
Even with normal matter and dark matter combined, though, we haven’t gotten close to finding everything. The remaining ~68 percent of the universe is unaccounted for, and our big clue toward what that “stuff” is first came in the 1990s, when observations of distant supernovae appeared fainter than our models of the universe were predicting. It was as though something else beyond what we expected — various forms of matter and radiation — was contributing to the universe. As the evidence poured in, bolstered by the cosmic microwave background and large-scale clustering data, we realized that a wholly novel form of energy, inconsistent with the properties of any form of matter or radiation, must be present: what we call dark energy today.
What is remarkable about the evidence for dark energy is how perfectly uniform it is. There is no evidence that there’s more or less dark energy in the space occupied by rich galaxy clusters than in the voids of empty space. There is no evidence that dark energy correlates with density, direction, location, or epoch of the universe. It appears to be perfectly uniform, perfectly homogeneous, and perfectly constant: unchanging throughout space and time. And yet, despite its simplicity, it behaves fundamentally differently from all other known forms of energy.
Various components of and contributors to the universe’s energy density, and when they might dominate. Note that radiation is dominant over matter for roughly the first 9,000 years, then matter dominates, and finally, a cosmological constant emerges. (The others do not exist in appreciable amounts.) However, dark energy may not be a cosmological constant, exactly. (Credit: E. Siegel / Beyond the Galaxy)
Every form of matter and radiation in the universe is linked to quantum particles in some way. Normal matter is made up of subatomic particles: particles of which there are a finite number. As the universe expands, the number of particles stays the same while the volume increases, hence matter gets less dense as time marches forward. Similarly, radiation is quantized into particles as well (even, theoretically, gravitational radiation, which should be quantized into gravitons), but these particles are massless. As the universe expands, not only does the number of particles remain the same while the volume increases, but the energy of each individual particle decreases as the universe expands.
Still, both of these descriptions fall apart for dark energy. As the volume of the universe increases — as it expands — the energy density does not change; it remains constant. It’s as though there is something present through all of space that isn’t dependent on anything else: matter density, radiation density, temperature, changes in volume, etc. Although we can measure and quantify its effects on the universe, we cannot say that we understand dark energy’s nature. It could be a
- particle of some type,
- a field that permeates the universe,
- or even a property inherent to the fabric of space itself.
A universe with dark energy (red), a universe with large inhomogeneity energy (blue), and a critical, dark energy-free universe (green). Note that the blue line behaves differently from dark energy. New ideas should make different, observably testable predictions from the other leading ideas. And ideas which have failed those observational tests should be abandoned once they reach the point of absurdity. (Credit: Gabor Racz et al., 2017)
Of course, each of these scenarios leads to a vastly different conception of the universe and what is present within it. If dark energy is a particle, then either new particles must constantly be created to keep the energy density constant, or the behavior of these particles must evolve with time to keep their effects on the universe constant. If dark energy is a field that permeates the universe, then it is permitted to evolve in either space or time or both, and any observed evidence (we have none) of such a variation would point in this direction; models of quintessence fall into this category.
But if we follow the observations, there is no evidence that dark energy is anything other than the most basic entity imaginable: a property that is uniformly inherent to space everywhere and at all times. This can come about in one of two different ways very easily:
- The universe can possess a positive, non-zero cosmological constant, a term perfectly allowable in general relativity. It has to be very, very small, but when you put it in everywhere over the whole universe, it eventually comes to dominate.
- It could be a quantum property of space: the zero-point energy of all the fields in the vacuum of space is not required to be zero but could take on some positive, non-zero value. What we often interpret as quantum fluctuations, or particle-antiparticle pairs popping in and out of existence, could be the cause behind dark energy.
Visualization of a quantum field theory calculation showing virtual particles in the quantum vacuum (specifically, for the strong interactions). Even in empty space, this vacuum energy is non-zero. (Credit: Derek Leinweber)
From a theoretical perspective, it is important to keep in mind that until we understand the nature of dark energy, which is to say that we acquire some sort of evidence that points toward one possibility over another, we have to keep all of these options in mind. Dark energy could be linked to the inflationary epoch that set up and gave rise to the Big Bang; dark energy could have been important and impactful early on in the universe’s history before decaying to its present, low-density state; dark energy could be slowly evolving or inhomogeneous, or could have a slightly higher or lower density dependent on what else is around. Theoretically, all options remain on the table.
But that is also why we don’t simply base our conclusions on theory alone. The whole idea of science is based on the notion that the way we find out information about the universe is by putting the universe itself to the test: through measurement, experiment, and observation. As we study:
- the cosmic microwave background down to smaller and smaller scales, in more wavelength bands and with polarization included;
- the large-scale structure of the universe out to greater distances, fainter objects, and larger areas on the sky;
- and individually luminous objects, out to greater precision and greater distances,
we gain the ability to see whether there is any indication that dark energy is anything other than a pure constant, and whether it shows evidence for any evolution or inhomogeneities in time and/or space.
This snippet from a structure-formation simulation, with the expansion of the universe scaled out, represents billions of years of gravitational growth in a dark matter-rich universe. Even though the universe is expanding, the individual, bound objects within it no longer expand. Their sizes, however, may be impacted by the expansion; we do not know for certain. (Credit: Ralf Kahler and Tom Abel (KIPAC) / Oliver Hahn)
Of course, it hasn’t. Fifteen years ago, we were able to constrain that dark energy was a constant to a precision of ±30 percent or so. Today, that has improved to a precision of ±7 percent or so, with the next generation of space-based and ground-based observatories — particularly ESA’s Euclid, NSF’s Vera Rubin observatory, and NASA’s Nancy Roman telescope — poised to take us to a precision of just ±1 percent. If there are any imperfections, inhomogeneities, or evolutionary effects that occur in the dark energy sector, these upcoming surveys will be our best bet at uncovering them.
However, there are other methods that could reveal some more exotic interpretations. Recently, the XENON experiment claimed to see an excess of events over the anticipated background, beyond what conventional sources could explain. There are three main interpretations on the table, at present:
- the result is an experimental fluke that will go away with better statistics, which is within the realm of possibility;
- that this is our first evidence of an unexpected type of dark matter, whose explanations would require additional contortions over what was theorized previously; or
- a new source of background that hasn’t been included in the analysis (such as tritium in the water) is causing it.
Of these explanations, most physicists favor the last one. But, as we said earlier, all possibilities, no matter how exotic or strange, have to be kept in mind.
That is where, as was recently shown by a small team of scientists, the idea of chameleon dark energy comes into play. If dark energy is actually a very specific, exotic type of particle that has its clumping and density restricted in the most matter-rich regions of space, it could have potentially created the signal seen by the XENON experiment. With some additional theoretical contortions, the team was able to conclude, at the ~95 percent confidence level, that this interpretation is favored over the null hypothesis: that the result is a mere fluke.
Of course, what most people do not realize is that this is precisely what most ideas in theoretical physics look like: you add in one or two new free parameters to explain one or two new phenomena. Most ideas like this are not new, but rather are variations on an old idea, and most ideas in this vein are colossally bad: they are ill-motivated and are considered only because experiments are nearing the precision necessary to rule them out, either wholly or at least in part. In physics, as well, a signal at ~95 percent confidence is barely worth a second look; this idea of chameleon dark energy particles likely will never rear its head again in any experimental setting.
Constraints on the total matter content (normal + dark, x-axis) and dark energy density (y-axis) from three independent sources: supernovae, the CMB (cosmic microwave background), and BAO (which is a wiggly feature seen in the correlations of large-scale structure). Note that even without supernovae, we would need dark energy for certain, and also that there are uncertainties and degeneracies between the amount of dark matter and dark energy that we would need to accurately describe our universe. (Credit: Supernova Cosmology Project, Amanullah et al., ApJ, 2010.)
When you take away wishful thinking and look only at the evidence that we have, the story the universe tells is very simple, albeit counterintuitive. The stuff that we thoroughly understand — the matter and radiation composed of all the known particles of the Standard Model plus gravitational waves — makes up only 5 percent of the total of what’s out there. There is another form of mass, dark matter, that makes up an additional ~27 percent or so. But the majority of what is present, the ~68 percent of the universe that is dark energy, doesn’t appear to either be a particle or change with time. It behaves neither as a particle nor as a field, but rather as a property that is inherent to space itself.
Although it is a fun exercise to consider what might happen under a variety of exotic conditions, particularly when experiments or observations are reaching the sensitivities necessary to probe them, it is vital to treat them as the fringe hypotheses that they are. The default, working hypothesis of what dark energy is doesn’t include extra couplings, clumpiness, time-or-space evolution, or anything else beyond a simple constant in space. It is time to take seriously the idea that dark energy might simply be a property inherent to the very fabric of space. Until we learn how to calculate the zero-point energy of empty space itself, or gain some bizarre, surprising, and unanticipated evidence, this will remain one of the biggest existential questions in all the universe.
Ethan Siegel is a Ph.D. astrophysicist and author of "Starts with a Bang!" He is a science communicator, who professes physics and astronomy at various colleges. He has won numerous awards for science writing since 2008 for his blog, including the award for best science blog by the Institute of Physics. His two books "Treknology: The Science of Star Trek from Tricorders to Warp Drive" and "Beyond the Galaxy: How humanity looked beyond our Milky Way and discovered the entire Universe" are available for purchase at Amazon. Follow him on Twitter @startswithabang.
Get Big Think in Your Inbox
Subscribe for counterintuitive, surprising, and impactful stories delivered to your inbox every Thursday.